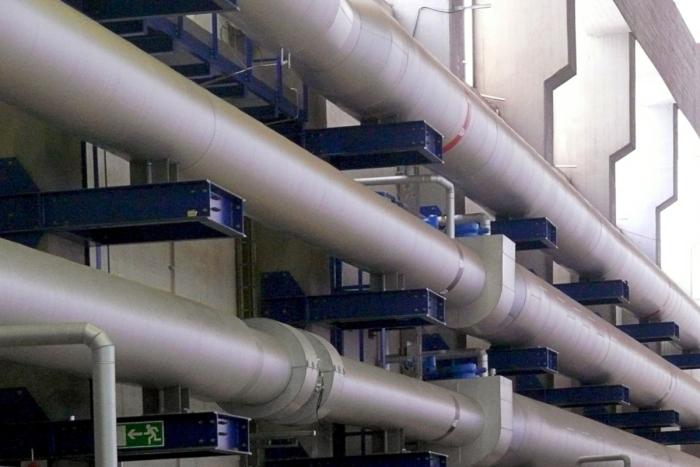
Details
Solution type
To foresee rapid changes in consumption and weather conditions and adjust the delivery in a district heating (and cooling) system with an inherent inertia is quite an art to master. There are some strategies and tools that are developed for this reason, to aid in optimising district heating and cooling on the utility-side.
The performance (economic, social, environmental and technical) of the DH/DC network can be improved by optimising its operation. Within the network, the utility side comprises all of the network up to the end-user substation. The aim is to operate the production and transmission/distribution facilities to reach high performance. There are several methods that can help in this process, and three of these methods are presented in this article. First, thermal energy storage enables you to decouple the production of heating/cooling for their use. Then the hourly scheduling of the production assets can be designed to be coordinated with the dispatch of heating/cooling, in order to improve the performance. Finally, increasing the integration of thermal energy services with electricity services can yield significant economic and energy savings through active network management and demand response.
Smart control integration of hybrid heating systems with Thermal Energy Storage (TES) in current energy systems offers great potential to integrate different energy sources and thermal energy generators to provide heating or cooling directly to end-users or as district heating or cooling (by thermal energy networks). The grade of success of this integration, measured commonly in CO2 reductions, energy efficiency and cost of thermal energy production, will depend on several factors: availability of resources, type of technology utilized, size of the TES, electricity prices on the project region, smart integration of the energy sources, etc.
The smart integration of the energy sources, energy generators and TES is essential for current and future individual or district heating/cooling systems. The smart integration of these technologies, as well as the interaction with other factors as the liberal electricity market, provides the possibility to integrate renewable energy and waste heat, allowing free cooling in a more efficient way. It can help in reducing the size of the heat generators, improve heat plant economics and more.
In the following sections, both individual heating systems and district heating integration will be described briefly.
Smart heat storage for solar heating systems
Heat supply (hot water and space heating) for buildings represents about 43% of the final energy consumption in the European Union (D. Andrews, 2012). In a future scenario with high shares of renewable energy sources, intermittent heat and power generation is expected to increase.
Figure 1: Smart heat storage for solar heating systems
During the first semester in 2014 in Denmark, 41% of the electricity consumed came from wind farms and it is expected that by 2050 about 50% of the increase in electricity consumed (including eg. transport and heat pumps) will be provided by wind power. In the heating sector, it is expected that 15% of the decreasing heat demand will be provided by solar heating by 2030, and up to 40% by 2050. From this last figure of 100% solar share, 80% will be provided by solar heating plants and the remaining 20% by domestic individual heating systems (Furbo, 2014).
Some of the problems related to these scenarios are the increasing mismatch between supply and demand and the increasing fluctuations of electricity prices on the energy markets. A solution already being implemented in Denmark to tackle this problem is the combination of different energy generation technologies, using smart heat storage to integrate them, and making the heating plant interact with the electricity grid (see Figure 1).
With this concept, solar district heating uses large-scale water pit energy storage (PTES). At the Dronninglund plant, the PTES has a capacity of 62000 m3 of water. In 2014 it was the largest of its kind in the world.
A similar integration of technologies is presented in the Marstal heating plant, a case study about the Marstal SUNSTORE 4. A description of this case study can be found in chapter 5 in Celsius deliverable D5.6: Concepts for storage and load control.
Smart solar tanks in combi systems for one-family house
The Roadmap Europe 2050 projects a 30% increase in district heating in Europe by 2030 and a 50% increase by 2050. Northern European countries such as Sweden and Denmark use high shares of thermal energy networks. However, there is still a long way to go before widespread thermal energy networks in Europe will exist in locations far away from dense urban areas where individual heating/cooling systems might be more economically feasible as a thermal network supply.
It is expected that solar and wind energy will cover most of the energy demand in future energy systems. The fluctuating power coming from wind farms will increase and periods with electricity surplus will be more frequent. A concept which integrates both solar heating systems and electricity power coming from wind is intended to increase the use of these two renewable energy sources.
Figure 2: The solar combi system layout. It shows solar collector, auxiliary heater, storage tank and hot water and heating loads in the house (R. Heimrath, 2007).
In these types of heating systems heat is produced by solar thermal collectors in combination with electric heating elements - or in combination with heat pumps, which are also driven by electricity (Figure 2). Electric heat devices and heat pumps operate when the heat demand cannot be fully covered by solar thermal collectors and when electricity prices are low. The system uses a smart tank (with variable auxiliary volume) as well as a smart control system. Therefore, heat losses from the tank are minimized and overall performance is increased compared to traditional systems.
Other kinds of smart solar storage using latent heat are being developed and investigated by the Technical University of Denmark (DTU). These systems are tested for seasonal storage and cover the heat demand of one-family house. The investigations are carried out with 9m2 surface area and tanks with water capacity of 735 l. Part of the heat demand is produced by solar thermal collectors on the roof of a single house, the rest is produced by heating elements or heat pumps. As solar heating cannot cover 100% of domestic heat demand, auxiliary electric heating units may be programmed to supply the rest of the heat when high shares of wind power is generated (causing low electricity prices). A complete description of this technology is found in Halvgaard et.al’s paper (2012).
More detailed discussions about the smart control system and its impact can be found in Chapter 5.1 of D5.3 Concepts for intelligent system integration.
Smart Water Heaters as Grid Batteries
Electric-powered water heaters and space heaters have a lot of virtual energy storage capacity and they’re already used in millions of homes and businesses. That makes them a cheap resource for grid storage if they can be managed in a way that allows them to respond to the grid’s needs, while still keeping the building at the right temperature (John, 2014).
In North America for instance, so called “Grid-interactive electric thermal storage” has been commercially developed for domestic hot water and space heating (Steffes, 2010). These devices help to solve the needs of today’s smart grids, such as variable generation, variable demand and variable prices (Steffes, Cost Effective Demand Response to Integrate Variable Resources and Provide Low Carbon Fast Regulation).
Figure 3: Three-year average of typical and smart water heater loads and usable wind
The system consists of a smart water tank with electrical resistances and smart control. The smart control of these combined systems decides when it is more efficient to charge the thermal storage. This decision is done according to three main aspects (see Figure 3):
- Electric load demand
- Real-time renewable energy production (wind and solar)
- Real-time pricing (RTP)
Electricity is stored as heat in water tanks or well-insulated brick cores, depending on the unit used. Storage occurs based on the availability of renewable or off-peak energy or as signalled by the utility for ancillary services. With smart control, the grid is affected like other electric storage technologies. The equipment uses an onboard microprocessor-based control system to regulate charging and discharging.
Thermal Energy Storage and Heat Pumps
Heat pumps provide space heating and hot water or cooling for residential, non-residential and industrial applications in all European countries. Heat pumps are a promising technology for load management in the built environment, in combination with the smart grid concept and thermal energy storage. Heat pumps can be coupled with thermal energy storage (TES) systems and shift electrical loads to avoid potential stress in power grids, integrate reluctant renewable energy (e.g. wind solar), or to produce cheap heat. TES can retain thermal energy by storing hot or cold water, or even other materials, for a period of time and release this thermal energy again whenever it is needed.
Heat pumps - Technical advantages and load control
Thermal energy storage brings environmental benefits, as it saves energy and contributes to climate change mitigation. Moreover, linking TES with heat pumps can be used to manage electrical loads in dwellings or buildings with heating or cooling demands. This mechanism can be applied for peak shaving or load shifting from on-peak when the price of electricity is high (typically during the day) to off-peak hours when electricity is cheaper (in most cases during the night). In this way, they serve as a powerful tool in demand-side management (DSM) strategies (A. Arteconi, 2012).
Some studies have shown that heat pumps together with district heating systems are the best solution for heat supply in existing and future energy systems (Hedegaard, 2013). The importance of the possibilities that these systems will provide in future energy systems is mentioned by Hedegaard, K., & Münster “the use of individual heat pumps is expected to grow considerably in Denmark, thereby developing into a significant part of the total electricity demand. The electricity demand of the heat pumps becomes flexible if e.g. investing in control equipment, enabling intelligent heat storage in the building structure and/or in existing hot water tanks, and/or if investing in heat accumulation tanks for space heating”.
Balancing the network is a very important technical aspect requiring the consideration of heat pumps and TES. It comes from the increasing penetration of fluctuating renewable energy. As Hewitt (Hewitt, 2012) has stated: “If a large amount of wind energy is to be superimposed onto a traditional fossil fuel-fired (or future nuclear) electricity network, the inherent smoothing associated with wind turbines widely dispersed across a geographical region may not be sufficient to manage the dynamics of existing fossil fuel and nuclear plant (in ramping up or down depending on network requirements). Therefore, an element of storage is required.” The technical university of Denmark (DTU) has carried out several studies on this aspect of balancing the fluctuating renewable energy with the energy demand.
Renewable energy integration with heat pumps and thermal energy storage into district heating systems
Denmark is strongly pushing forward towards a 100% renewable supply of energy by 2050. One radical measure taken in April 2013 was the banning of oil or natural gas heating systems in new buildings. The measure will become more extreme in the future, as even existing oil heating systems are to be changed if there is a local supply of district heat or natural gas available by 2016 (Energy transition - not just electricity, 2013). In order to achieve this goal “Research work at the Technical University of Denmark aims to find out ways to couple the excess production of wind energy with heat pumps and thermal energy storage, so as to replace a large portion of the thermal energy demand – otherwise met by fossil fuels – in the residential and commercial sectors” and they claim also “we have found that it would be economically feasible to replace almost all the fossil-fuel boilers with a combination of a heat pump driven by renewable electricity and an energy storage system” (H. Hvidtfeldt Larsen, 2013).
Heat pumps show significant potential to integrate renewable energy when successfully coupled with thermal energy storage. The performance of the system can also increase and thus decouple energy supply from energy generation, allowing a reduction in the heat pump’s size. Furthermore, it is possible to reduce operational costs by taking advantage of the off-peak electricity tariff – as well as to avoid power stress in the electricity grid in the case of the residential heat pumps. Another benefit of heat storage is that it can operate as a backup system when a problem occurs with the heating system.
Integration of heat pumps and Thermal Energy Storage into current district heating systems
Heat pumps linked to thermal energy storage are already part of district heating systems and, together with other energy sources, satisfy the heat demand of entire districts in several cities. Using large-scale heat pumps in district heating networks is quite common in for example, Denmark and Sweden. Some of the reasons for using large-scale heat pumps instead of residential heat pumps are that "Central heat pumps are more efficient, more compact, cheaper and deliver higher temperatures than individual heat pumps" (D. Andrews, 2012).
Figure 4: Principle hydronic system of SUNSTORE 4 (Hammerschmid, u.d.)
A good example of the utilization of heat storage (in combination with heat pumps and other technologies) as solar thermal collectors is the plant at Marstal, Denmark.
As part of the solar heating plant, the thermal energy storage in Marstal is used all year long, due to its large size. The pit thermal energy storage (PTES) is mainly used to store the warm water coming from a solar thermal collector field. The PTES at Marstal is an excavated pit, which is lined by a membrane and filled with 75,000 m3 of water (T. Schmidt). The operation temperatures range from 30 to 90C and its dimensions are the following: 113 m length, 88 m width and 16 m depth. The storage is not insulated towards the bottom and the walls of the excavated pit since the losses through the soil are low. The top of the storage, on the other hand, is insulated and covered by a floating liner (PLANENERGI, 2013).
The PTES allows for the integration and storage of hot water coming from the solar field. The hot water is integrated at different heights depending on its temperature. The entire heat demand is covered by the solar plant and the surplus of solar energy harvest is preserved until winter. It also provides the input for the heat pump throughout the year.
The heat pump has a power of 1.5 MWth and uses CO2 as a refrigerant in its multi-compressor system. Its COP (coefficient of performance) is very sensible on the inlet temperature on the hot water circuit (which corresponds with the gas cooler outlet temperature) but can reach high hot water outlet temperatures (~ 80°C) without a reduction of COP. These temperatures are inlet 35°C and outlet 80°C (Hammerschmid).
Some of the features that the heat pump provides to the solar plant are:
- Utilizing low-temperature energy from the bottom of the heat storage
- Heat capacity of the pit heat storage will be increased due to a larger temperature difference
- Efficiency of the solar system will be increased due to lower inlet temperature from the bottom of the pit heat storage
- Reduction of heat losses in the pit heat storage due to lower average temperature
- The operation of the heat pump takes place mainly at the end of the winter season in order to optimise the solar efficiency and the solar yields
- The heat pump provides the flexibility of electricity consumption, thus it produces heat during the winter when prices are more convenient for its operation
For more details about heat pumps in Denmark and Sweden and about this project, more information and a case study can be found in the D5.6 Concept of Storage and Load Control.
Thermal Energy Storage and CHP
By capturing the waste heat associated with electricity production, combined heat and power plants (CHP) can produce electricity and heat at the same time, thus reducing operational costs, emissions and primary energy consumption, making them more attractive than conventional separated heating plants for sustainable energy generation. CHP can meet the electricity and heat demands of a commercial building (e.g. hotels, schools, offices, restaurants, hospitals, supermarkets) or it can be connected directly to the power grid and supply a district heating network. However, a common concern is the mismatch between electricity and heat production and electricity and heat demand.
The relation between the electric and heat load demand is called power-to-heat ratio, so CHP installed in buildings with low power-to-heat ratios tend to have a bigger excess of heat production. This excess of heat can be addressed by coupling the CHP generator to a TES system so that the heat can be stored and used later when it is needed. If heat supply and demand are better managed, the overall efficiency and economics of the system can increase.
One opportunity to optimise CHP operational performance, energy production, and electricity demand is for example in the UK and northern Europe, where these power plants provide the so-called “base load heat demand”. In this case, there are some periods when the production of electricity is limited by the production of heat because it is not needed at the moment. Coupling TES to CHP plants allows for storing heat when producing electricity is economically attractive even tough heat is not necessary.
Adding a TES to the CHP plant brings more operation flexibility and allows the plant to operate longer during the year. These benefits can solve problems for CHP plants in current and future energy systems, such as decreasing stock market prices for energy and decreasing heat demand. The profitability of adding large thermal energy storage to an existing district heating system in Germany with different heat generation technologies is identified by Groß et al (S. Groß, 2014). Groß also points out that “Combined heat and power-based district heating is a changeover technology on the way to Germany’s full energy supply through renewable energies”.
Control Strategies for micro CHP coupled with Thermal Energy Storage applicable in District Heating Systems
Different control strategies are available in operation for micro CHP coupled with Thermal Energy Storage. A simulation tool was developed by the University of Applied Science in Cologne (Germany), where they linked a CHP and TES, in order to show the different possibilities to control the operation of a small CHP plant when it is coupled with thermal energy storage. This way, you can see how flexible the electricity and heat production of the CHP can become in winter and summer when heat demand varies greatly.
For a detailed discussion and analysis, please refer to section 5.3.1 in D5.3.
Opportunities for new control designs for increased renewable energy integration and recovery of waste heat recovery
It is common to connect a CHP to a district heating network. A combination of all mentioned control strategies provides yet more possibilities for efficient management of the system.
In addition to the CHP system - which is connected to the district heating network - a storage system waste heat plant could also provide heat, charge the storage or supply the network. Several options are possible. Thermal storage installed nearby to the CHP or networks enable the use of waste heat or peak shaving methods. One possible scenario for the summer period could be to connect the waste heat plant to a heat pump (HT) to charge the store while the CHP is switched off - as the heat demand is shrunk to the demand of domestic hot water during the summer. Depending on the primary use of the CHP you could also do the reverse. Another possible scenario is to load the store during the night and provide additional heat during morning peaks in order to shorten the operation period of peak boilers, or even to turn off the boilers completely.
Demand Forecasting and Dispatch Optimisation at Warmtebedrijf Rotterdam
Warmtebedrijf Rotterdam (WBR, Dutch for "Heating Company Rotterdam") is an energy company that has provided transport of residual heat, derived from a waste incinerator, to the district heating networks in the city of Rotterdam since October 1, 2013. Formally WBR consists of two companies: WBR Infra NV owns and maintains the network. Its shareholders are the City of Rotterdam (88%), Woonbron (4%) and the Province of Zuid Holland (8%).WBR Exploitatie NV is responsible for the commercial exploitation and delivery of heat. The shareholders of WBR Exploitatie NV are the energy company E.ON Benelux (50%) and the City of Rotterdam (50%).
WBR owns and operates the transport district heating network in Southern Rotterdam, which is connected to the Northern Rotterdam network (owned by Eneco), at the Heat hub at node 4 in Figure 5 below. WBR's main energy generator is the waste incinerator at AVR Rozenburg. A total of 105 MWth thermal capacity is available for WBR. As WBR is actively discussing opportunities with other suppliers of waste heat in the Rotterdam Harbour area, the total available capacity to WBR is expected to increase in the coming years.
Figure 5: The district heating network of Southern Rotterdam, owned by WBR
WBR does not directly supply heat to residential customers. In Southern Rotterdam, it delivers heat to the local distributor Nuon, which then supplies it to its customers (residential buildings, hospitals, etc.). In Northern Rotterdam, WBR delivers heat to the distribution company Eneco and any excess heat that WBR has available is delivered to E.ON.
As further assets/objects, WBR owns physical heat storage with a capacity of 185 MWh at the Heat Hub. It is common practice to place a buffer next to a production facility where it serves as pressure control. The buffer at the Heat hub does not have this function and was strategically placed in order to optimise the capacity of the distribution network and serve as backup capacity. Furthermore, there are several booster stations for regulating the pressure in the distribution network. WBR also owns the heat storage at AVR, which has a capacity of 270 MWh. This device maintains and controls the pressure in the WBR network but is also used for economic optimisation.
Figure 6: The heat storage of the Heat Hub located at the Brielselaan
WBR’s primary responsibility is to continuously supply heat to its customers in Rotterdam. In Southern Rotterdam, WBR is the sole supplier of heat into the distribution network. In Northern Rotterdam, next to WBR, E.ON and recently Eneco also supply heat to the distribution network. E.ON owns the conventional production assets in Northern Rotterdam. The assets owned by E.ON include several boilers, gas turbines, a CHP and heat storage.
WBR and E.ON perform the dispatch of district heating of both Northern and Southern Rotterdam together. This means that the dispatch is jointly optimised. In practice, this means that heat from the more efficient (from an economic and environmental perspective) WBR waste incinerator is used to meet demand in Northern Rotterdam instead of heat supplied by the conventional assets of E.ON.
The joint production of heat and power together with the variability in the power price, the cost of production and the opportunity to use the heat stores constitutes a mathematical optimisation problem. The price of heat is constant/exogenous in the optimisation horizon (of a week), so the optimisation objective function is to maximize the power revenues, minus the production costs of both power and the heat of the combined Northern and Southern part of Rotterdam.
Forecasts of the prices of power, gas and emission rights are input for the dispatch optimisation. Forecasts of the heat demand, which is dependent on weather and behavioural and seasonal factors, are input for the dispatch optimisation as well. Heat demand is measured per district and it enters the dispatch optimisation at an hourly interval.
The measured data by WBR and other relevant data that is collected for the dispatch optimisation process are described in the first section below.
Data and Measurement
Measurement of demand data occurs at a district level. Variables such as flow, flow temperatures, return temperature and supplied heat are measured at various points in the network. The heat is measured and calculated by compatible flow meters (e.g. Figure 7) and temperature sensors. The variables are measured continuously and read out on a quarterly basis.
Figure 7: One of the meters
Figure 8: The Energy ICT portal
The heat-related data is available through an online portal called Energy ICT (see Figure 8). This portal can be used for real-time observation and decision-making. For more long-term analysis measured data are exported through ftp to a more extensive data-warehouse environment.
Other data that are necessary for the dispatch optimisation are collected in the data warehouse as well. These include weather data for making heat demand forecasts and the market prices of power, gas and carbon.
With this historic data, forecasting of demand and prices is done for the coming week. Based on this forecasted data, dispatch is optimised. The optimisation process is repeated daily, for the next day following it, with newly available data. The dispatch optimisation, and the tool that is developed for it, are reviewed in the following section.
Dispatch Optimisation
Background
E.ON and the WBR share responsibility for the dispatch of the district heating of the Rotterdam area (See Figure 9 for a network representation). A joint optimisation is performed for both the Northern and Southern Rotterdam areas.
The heat-demand districts that WBR supplies are the Hoogvliet, Maasstadziekenhuis, Brielselaan and Eneco-Noord districts, and E.ON’s are the Stad-Kop van Zuid and Bdriehoek-Capelle districts. WBR supplies its own Southern districts and the remainder is sent over from the "Heat-hub" to the Brielselaan on the North side. As AVR is the most efficient generator, it is used most of the time and supplies a considerable part of the North side.
Figure 9: An operator screen displaying a representation of the network.]
Other assets for the production of heat are the ROCA-1 and ROCA-2 (gas turbines), the ROCA-3 (a CHP), the boilers of WSG, Blekerstraat, Delftsevaart-KopVanZuid and ROCA. These production assets use gas as fuel and emit carbon dioxide.
Furthermore, there are the AVR, WBR and WSG heat stores, which can both be used for economic optimisation and as backups in case of failure.
The selling price of the heat demand for WBR does not vary in the optimisation horizon of a week, so the revenues of heat demand are not taken up in the objective function of the dispatch optimisation, and therefore the objective function reduces to maximize the power revenues minus the costs of heat generation for the Rotterdam districts and the generated power. This mathematical optimisation is performed with AIMMS, which is a mathematical modelling and optimisation tool.
The dispatch optimisation is performed daily and for a week ahead. While this is a theoretical optimisation, which is based on forecasts, and practice is always different, operators are standing by for necessary adjustments.
The dispatch optimisation tool is updated if new information about the production assets or heat stores becomes available. When a new asset or demand district is added, or the network structure is changed, a redesign of the tool is necessary.
Necessary input for the dispatch optimisation tool
The optimisation tool needs the following parameters as input:
- The characteristics of the production assets such as producible heat and power combinations and their fuel usage, availabilities, ramp ups and ramp downs, minimum up and down times, some history on and off, and other additional production costs.
- Characteristics of the heat stores such as start and end levels, maximum storage levels, availability and heat losses.
- Network characteristics such as pipe capacities and heat losses.
- The forecasted hourly values of the price of power, gas and carbon, and the forecasted hourly values of demand per district, (see the paragraph below for more in-depth comment).
Solving by the Dispatch Optimisation Tool
The dispatch optimisation tool was developed in 2013 in the modelling environment AIMMS 3.13. Besides solving, AIMMS has the advantage of model visualization and comprehensive data presentation (see Figure 10).
AIMMS has a variety of solvers to solve Linear and Integer Programming problems. As there are a number of on/off decisions in this situation, the type of problem is a mixed-integer program (MIP). CPlex 12.5, which is a very common solver, is used to solve this MIP.
The objective function of the dispatch optimisation tool is to maximize the power revenues minus the cost of power and the heat generation.
The main restrictions for the optimisation problem, in terms of equations and inequalities, are the restrictions of the pipe capacities and their losses, the production restrictions of the assets, the restrictions of the heat stores, and the hourly demand to be supplied to the districts.
When making a few assumptions on production restrictions, it is possible to find the optimal solution within an hour of solving time on standard laptops.
Output produced by the Dispatch Optimisation Tool
The output of the dispatch optimisation tool can be summarized by:
- The value of the objective function (and its breakdown in hourly power revenues and production costs).
- The hourly production scheme of the assets.
- The behaviour of the heat stores.
- The hourly flows of heat over the pipe network.
In the next section, an overview of the forecasting process is given.
[caption id="attachment_6263" align="alignnone" width="673"]
Figure 10: Screenshot of Network used in 2014 within the AIMMS optimisation module.
Forecasting
For the dispatch optimisation tool, a number of forecasted values are required. These can be categorized in the prices of power, gas and carbon, and the heat quantity required to meet the demand in the different districts. Power is a gain that comes as an additional product from the heat production process, gas is a cost that is made in the production process of some assets, and "carbon" are the costs for the rights to emit the carbon that comes free from gas-firing. The forecasted heat demand is important for production planning, and for the quantities of heat that E.ON on the North side of the Heat-hub can expect from WBR.
The values for the forecasted variables enter the mathematical optimisation problem at an hourly level.
Price Forecasting
The main method for forecasting the spot prices of power, gas and carbon is to estimate a week-shape of these prices out of a representative history, where the market structure is comparable to the current market. The week-shape is found by inspecting the relative behaviour of market prices within a week and then averaging this behaviour over the entire period of history. In the final step, this obtained (average) week shape is fitted into the available forward prices for the upcoming period/horizon. In Table 1 an overview of the used spot and future data for the forecasts is given.
Table 1. Sources of data for forecasting
Demand Forecasting
As every district has its own building types and their users have different purposes and behaviour heat demand is forecasted per district.
For heat demand forecasting, a history of hourly heat consumption per district, and a history of weather-related data for this area are necessary. The aim is to estimate a relation between heat consumption and weather-related variables. In this relation, the weather-related variables are the explanatory variables and heat consumption is dependent. As weather-related variables temperature, wind, sunshine, clouds and humidity are taken up. The data of these variables for the Rotterdam area are obtained from Meteo Consulting.
Since the start of WBR on October 1, 2013, a regression model has been used to estimate this relation for every week-hour with an extra stratification on self-defined seasons within a year. The main method has been to relate heat consumption to a cubic polynomial in experienced temperature, which is extracted from wind and temperature, and the addition of a subset of the remaining weather variables.
While this method was sufficient for its purposes in the start-up period, WBR is currently improving on this by remodelling heat demand forecasting with a neural network model, which is planned to be ready by March 2015.
Active Network Management and Demand Response at Islington London
UK Power Networks is trialling low-carbon innovation technologies and reshaping commercial processes, seeking the best solutions for integrating these into business as usual. As part of Low Carbon London, a four-year program of demonstrator trials, UK Power Networks trialled Demand Response (DR) extensively and is therefore well placed to develop this learning to find long-term solutions for the security of supply.
Brief description of the demonstrator
The role of UK Power Networks in this work package of the Celsius Project is to provide DR to alleviate network constraints and faults. At times of high network demand, the transformers in substations can reach loading levels outside of operational guidelines. While this is not necessarily dangerous, when a transformer has exceeded statutory limits it can lead to thermal and load faults. In both cases, the operation of the transformer will either automatically trip out or need to be temporarily suspended. If DR can be used to reduce the demand on the substation, reducing the loading - the number and magnitude of these faults may be reduced or avoided. Times of elevated loading are referred to as a “constraint”. When normal network running arrangements need to be reconfigured due to, for example, a damaged cable or blown fuse, this is referred to as a “fault”. In this case, the surrounding substations will need to meet any shortfall in electricity distribution, elevating their load levels, which can then result in them being constrained. This is where DR can help. By providing a supplementary supply within the local network, known as a Distributed Generation (DG) asset, the load on the substation can be reduced.
UK Power Networks will be working with Islington Council, which owns the Combined Heat and Power unit (CHP) at Bunhill Energy Centre (BEC), to contract DR services as part of the Celsius project. The aim of this exercise is to learn how best to incorporate CHP-generated electricity in times of network need. Along with operational learning, there will also be contractual learning, most notably from running BEC during the colder months when there is not the same flexibility as in warmer months.
The events will be autonomously dispatched via a system developed by UK Power Networks and Smarter Grid Solutions (SGS). This Active Network Management (ANM) system will initiate events as required by the network, triggered by a breach of the substation load threshold.
Technical Solution
Monitoring
There are several stages to undergo in order to set up a Demand Side Response (DSR) resource. UK Power Networks has undertaken monitoring of BEC to assess the capacity to parallel to the network at varying times, from a diurnal to an annual scale. In conjunction with this is the assessment of substation loading which will provide times at which DR may be needed.
ANM Interface
This monitoring could not have taken place without the ANM equipment in place. The ANM equipment is capable of monitoring substation loads and dispatching DR events when the network is in need. A diagram of the ANM interface is shown in Figure 11. Components and measurements are also detailed below.
Thresholds
Extensive monitoring of substation loads has been undertaken to find a suitable level at which to place the thresholds for event dispatch. Factored into the ANM configuration are variables which ensure events are not triggered unnecessarily. For example, when the threshold is breached momentarily, an event is not triggered until loading has exceeded this level for a certain time period. Similarly, when the load dips below the threshold, a lower threshold must be reached, and the load held below this for a certain period, before the event has ended.
Durations
The duration of each event depends on the trigger. If the network is under fault, then the event could be as long as technologically and contractually possible. If the event is due to high substation loading the event may be shorter, lasting until the period of elevated loading passes. Similarly, if the event is dispatched manually (still using the ANM interface but triggered using a Human Machine Interface (HMI) rather than substation load), the event may be last longer than normal as this scenario will usually be in the case of a severe network fault. In any case, events will typically not last longer than 3 hours, since the filling of the thermal store inhibits further use.
Commercial Aspects
There are a number of commercial agreements to be made to enable the use of BEC for Celsius in a way that benefits everyone involved. Current technological restrictions limit the length of events, but alterations can be made to the infrastructure to accommodate longer events, bringing value to the Distribution Network Operator (DNO) and increased learning of new contractual processes to benefit the Celsius Project. The possibility of longer-duration events is explored in the last section below.
Event Dispatch
In theory, there should be one method of event dispatch. ANM is the most future-proof as it is autonomous and reactive to the needs of the network. Once thresholds have been incorporated into the ANM system and changed when necessary (depending on the month or season and due to load fluctuations) there should be little human intervention. In a trial scenario, this is somewhat different. To test event responses (more specifically the capacity to respond at a certain time, for the duration required), events can be manually triggered. This still uses the ANM interface to send the dispatch signal; the trigger is not a breach of threshold, but a human choice and the signal is sent using an HMI. This will enable a higher number of events to take place in a trial period to maximize learning.
General Layout
Main components
The ANM infrastructure operates based on four sets of functional components collectively interacting at any point in time, as seen in Figure 11. These elements have a degree of autonomy whilst interacting with each other in a structured manner. The key operational element in ANM is the SGS power flow software application that resides in the SGS core hardware. SGS power flow is an autonomous algorithm that performs real-time management of energy-producing and consuming devices to maintain power flows within the constraints of the network.
The ANM components consist of:
- SGS core – This runs the SGS power flow application that performs ANM and collects data from and passes data to the SGS comms hub.
- SGS comms hub – Collects data from and passes data to SGS core, SGS connect, and Measurement Points (MP). The SGS comms hub also provides an interface to the UK Power Networks Operational Data Store (ODS) and to the UK Power Networks SCADA system.
- SGS connect – Provides an interface to the DG load native control system to regulate the import and export of energy from the DG load and ensure fail-safe operation of ANM.
- MP – This can be a value (or values) collected directly from a measurement device monitoring current and power flow at a single constraint location on the network, or from a Measurement Point Controller (MPC), which itself collects values from a measurement device.
- Primary/Local User Interface (UI) – User Interfaces are located at the Central Controller and each SGS connects. The Primary UI allows network operators to interact and supervise ANM | DR from the location of the central controller. The Local UIs allow network operators to interact with the relevant SGS connect.
Figure 11: ANM and DR outline design
Measurements available
The ANM system measures at the substation and at the DG asset. A Remote Terminal Unit (RTU) at the substation reads load values and the ANM box connected to the DG asset measures the output of the generator.
The measurements at the substation are:
- Real Power (kW)
- Max Real Power (kW)
- Real Power set point (thresholds) (kW)
- Reactive Power (kVAr)
- Reactive Power Set Point (thresholds) (kVAr)
- Voltage (V)
- Power Factor (-1 to 11)
- Max positive Power Factor (0 to 1)
- Max negative Power Factor (-1 to 0)
- Current (A)
- Major fault indication (I/O)
- Capacity available for ANM dispatch (kW)
Measurements at the DG asset are:
- DR availability (kW)
- Real Power (kW)
- Voltage (V)
- Current (A)
- Power Factor (-1 to 1)
- Reactive Power (kVar)
Expected impact on Energy Efficiency and the Environment
Quantifying the impact of BEC’s contribution to the Celsius project is no easy task. The emphasis is less on energy efficiency and more on the security of supply. The benefit of this increased security of supply, as well as the carbon impact, is entirely dependent on the number of events and their duration.
Energy indicators
The operation of BEC as a DR asset is intended to provide ancillary electricity supply to the network at times of peak demand when the loading on nearby City Road has reached levels where action is needed to protect the transformer(s) from constraint or fault. The most ideal situation is for BEC to be automatically dispatched at times of need so that the locally connected customers do not notice any change to their supply. This is the value case. Not only can UK Power Networks reduce their maintenance and equipment upgrade costs, potentially deferring this into the next regulatory price control period but they can also avoid paying fines to the industry regulator Ofgem, in instances of fault or outage.
Not accounting for losses, the effective drop in substation load should be equal to the output of BEC, approximately 2 MW. This could be argued as an energy efficiency measure as this much electricity is not drawn into the DNO network from the National Grid. It would, however, be lost in the noise due to its relative insignificance and would fail to register with electricity generators or procurers. If this technique were to be deployed on a larger scale, city-wide, then this would not be the case, but Decentralized Energy (DE) is less efficient than large-scale generation, resulting in a net increase in energy used. For this reason, one could argue there is little energy efficiency in the operation of BEC, but the benefits are the value to the customer through increased security of supply and cost savings through deferral of network upgrade.
Environmental indicators
Figure 12: Example events (electrical power) contributing to carbon footprint and the associated cost
Assessing the environmental impact of DR events at BEC is not simple and subject to the number of events, set against standard use. The normal operating hours will have to be assessed and any events which land outside these times will need to be quantified. It is these events which will contribute to a carbon assessment. An example of a CHP event is shown in Figure 12, portraying a typical event time and duration.
Figure 12: Example events (electrical power) contributing to carbon footprint and the associated cost.
Economy
The contractual aspects of BEC are still being defined by the London Borough of Islington. A summary of the envisaged payment structure and the cost to the DNO is given below.
Payment Structure
Through previous work with DR contracts in the Low Carbon London Project, UK Power Networks has offered payment to DR providers in the form of availability payments and utilization payments. The DR provider is paid an “availability payment” for the hours and days they are available to offer their services to the DNO. They are also paid a “utilization payment” for the hours they have supplied their service.
This method of payment is currently not economically sustainable and has only been used in trial settings. In the future a DR provider could theoretically be available every day of the year, resulting in a huge cost to the DNO which does not hold up well to the business case of customer benefit through cost saving.
It is envisaged in this project that the availability payments will be dropped, and the utilization payment will be of a higher value. Price modelling is currently in progress and has shown to be less wasteful. This holds up as future-proof while the DNO looks for more economically sustainable ways to increase the security of supply.
Customer Value
Customer value is one of the main drivers of the change in payment structure. If bills can be reduced (in real terms) while providing a more secure electricity supply going forward, then UK Power Networks has succeeded in one of its core values; to be “sustainably cost efficient”. If this can be achieved through the development of complex commercial and operational contracts with DR providers, the beneficial learning can be scaled-up to other areas in the DNO’s license areas, providing the same benefits.
DNO Value
Calculating the value of the DNO is a long and involved task which will be undertaken at a later date within Celsius. It can be summarized as follows:
- Deferral of network reinforcement through the value of ‘lost load,’ offsetting reinforcement and replacement costs through load reduction measures (DR events).
- The reduction in number and cost, or the avoidance of fines payable to Ofgem and customers through increased security of supply.
Although the figures in this example are not on par with those at City Road and BEC, the principle is the same. The current primary substation at Whiston Road (11 kV) is due to reach its peak load because of organic load growth in the area and will need upgrading to accommodate this. There is not enough space at the substation for the upgrade to take place - a new substation will need to be built and the load transferred. The new substation is proposed to be built in nearby Hoxton. The use of DR has been investigated at Whiston Road and found to enable UK Power Networks to defer the build of a new proposed substation out of RIIO ED1 while helping to manage the network constraint at Whiston Road. Contracting 5 MVA of DR between 2021 and 2025 (inclusive) for £293,640.00 will defer the new substation cost of £13,125,000, saving a total of £12,831,360.
Lesson learned
Invaluable lessons, both contractually and operationally, will be taken from events at BEC. As the relative cost of customer electricity bills increases, DNOs are working to provide security of supply in the most economically sustainable way. DNOs are entering a time of change, becoming Distribution System Operators (DSOs), which means they are actively controlling customer demand for mutual benefit. Contracts with Decentralized Generation (DG) providers of ancillary supply will become commonplace and by trialling and improving the service provided, incremental improvements can be made and built upon for future contracts with other DG providers. When scaled up, this form of energy efficiency and security of supply can provide real benefits to the DNO in the form of network reinforcement deferral and the avoidance of fines. At the same time, customers benefit from lower bills and a more secure supply.
Operationally speaking, there are many complicating factors to manage DR events. The number is greater when dealing with CHPs. For example, there are constraints on the duration of events due to the filling of thermal stores. Once full, the generator must turn off. This can be circumvented by retrofitting a heat dump, enabling the supply of ancillary electricity supply without filling the thermal store. Given the business case, the DNO may wish to contract this from the DG provider and this facility may well be something which is retrofitted to BEC if it has value. Network faults often last for many hours or even several days. If this ancillary supply can be contracted for at duration, there will be significant value to the DNO and its customers.
Related Celsius content:
How to plan and optimise the production of district heat
References:
- Arteconi, N. J. (2012). State of the art of thermal storage for demand-side management. Applied Energy, vol. 93, ss. 371-389.
- Andrews, A. R.-G. (2012). Background report on EU-27 district heating and cooling potentials, barriers, best practice and measures of promotion. Joint Research Centre, JRC Scientific and Policy Reports
- Energy transition - not just electricity. (2013). Renewables The Magazine International,.
- Furbo, s. (2014). Smart Heat Storage for solar heating systems.
- Hvidtfeldt Larsen, L. S. (2013). DTU international energy report 2013: energy storage options for future sustainable energy systems. Roskilde: Technical University of Denmark.
- Hammerschmid, A. (u.d.). CHP Plant based on a Hybrid Biomass and Solar System of the next Generation. EU Project No.ENER/FP7/249800/‘SUNSTORE 4. BIOENERGIESYSTEME GmbH.
- Hedegaard, K. &. (2013). Influence of individual heat pumps on wind power integration – Energy system investments and operation. Energy Conversion and Management, 75, ss. 673-684.
- Hewitt, N. J. (2012). Heat pumps and energy storage – The challenges of implementation. Applied Energy, vol. 89, no. 1, ss. 37-44.
- John, J. S. (2014). Aggregating Water Heaters as Grid Batteries: Steffes’ Secret Sauce. Hämtat från Greentech Media: https://www.greentechmedia.com/articles/read/aggregating-water-heaters-as-grid-batteries-steffes-secret-sauce
- PLANENERGI. (2013). Deliverable D 2 2 SUNSTORE 4 Design of the pit heat storage of the demonstration plant at MARSTAL FJERNVARME Version 3. Planenergi.
- Halvgaard, P. B. (2012). Model Predictive Control for a Smart Solar Tank Based on Weather and Consumption Forecasts. Energy Procedia, vol 30, ss. 270-278.
- Heimrath, M. H. (2007). Project Report A2 of Subtask A, the Reference Heating System, the Template Solar System. A Report of the IEA-SHC Task32.
- Groß, M. R. (2014). Large heat storages for load management in CHP-based district heating systems. The 14th International Symposium on District Heating and Cooling. Stockholm.
- Steffes, P. (2010). Smart storage – space and water heaters.
- Steffes, P. (u.d.). Cost Effective Demand Response to Integrate Variable Resources and Provide Low Carbon Fast Regulation.
- T. Schmidt, D. M. (u.d.). Large-scale heat storage. Eurosolar.
- Warmtebedrijf Rotterdam (WBR). (u.d.).
Replicability |
Low |
Medium |
High |
---|---|---|---|
Authorizative easiness | x | ||
Adaptability to different climate conditions | x | ||
Technology easy-to-implement (No needs of specific technical requirements) | x | ||
Easy-to-implement (No needs of specific technical requirements) | x | ||
Easy-to-operate (No needs of specific technical requirements) | x | ||
Opportunity of integrating waste energy sources | x | ||
CAPEX needed for the deployment of the solution | x |